Energy industries are constantly challenged to become ever more efficient and sustainable. Oil and gas producers are not exempt from these pressures, as squeezed margins and a rapidly evolving regulatory environment mandate increased efficiency in process/output as a core driver for adopting new and improved technologies. Common to both ‘greener’ energy sources like solar and green hydrogen, and oil and gas is minimizing downtime and OPEX (operational expenditure) via serviceable systems and reliable part supply, allowing the processes to keep running and maintain their highest levels of output possible.
Additive Manufacturing has promising applications in a large number of energy related applications: 3d printed battery electrodes and capacitors, as well as micro-structures that can control energy transfer, leading to solar panel surfaces and refractive coatings, new fuel cell design and photocatalytic reactors.
Heat transfer applications are also well suited to deriving significant benefits from additive manufacturing. The generation of, and subsequent transfer of heat, is a large part of the process in the creation of stored energy sources, so any advance in technology that lends the opportunity for greater efficiency is one to be explored and progressed. AM can offer a significant impact in energy industry due to its potential for increasing the efficiency of heat transfer or by intensifying this process so it can occur within a smaller or lighter heat exchanger.
Heat exchangers in energy applications
Energy applications typically involve high levels of hear transfer. This translates into one or more of extreme volume, pressure and/or temperature.
Heat exchangers in oil and gas or energy industries are used for key processes like cracking, atmospheric distillation and heat removal from numerous mechanical operations are typically very large, over 1m3 in size and up to 20m long in some circumstances. Commonly found heat exchangers include shell and tube style, plate style, tube or pipe. These traditionally manufacturing are relatively scalable as long as there is ample space, enabling design and build of very large units for high volumes of heat transfer – which is required in these industries.
The challenge of energy storage and the role of ‘green’ hydrogen
We can create greener energy, the next step we need to overcome is the storage of this energy so that it can be used how we want to. Green Hydrogen, the frontrunner for storing energy from sustainable sources, uses electricity from appropriate sources to split water molecules by way of electrolysis, extracting the hydrogen, and leaving only oxygen as the byproduct.
Storing and transporting hydrogen involve pressure and temperature changes and therefore heat transfer. The most common forms of hydrogen storage and transport currently involve:
- liquification at extremely low temperatures
- high pressure storage, typically between 350-700 bar
- chemical storage, leveraging metal hydrides, organic compounds or ammonia to convert and extract the hydrogen from a more energy dense and easily transportable form
- gas pipelines, often leveraging/converting existing natural gas infrastructure to accommodate hydrogen
To condense the hydrogen into liquid, where it can be used as the fuel, you need to cool it down via a multi-step process (the boiling point of hydrogen at one atmosphere pressure is -253°C). Transportation of liquefied hydrogen is complex, involving highly insulated or cooled cryogenic vessels and exacerbated losses due to slopping during transport. Once compressed hydrogen is ostensibly more transportable, but the density far less that of liquified hydrogen - 24 or 40 g/L compressed to 350 or 700 bar at room temperature vs 70 g/L for liquid H2 at 1 atm and -253°C. Highly compressed gasses also carry a risk of explosive decompression in an accident.
Distributed solutions that enable local condensing of hydrogen bypass the challenges of transportation, but require localized compression or cooling equipment and heat transfer. Commonly envisaged small scale users of hydrogen are; Hydrogen fuel cell electric vehicles (FCEVs), hydrogen heat pumps for residential and commercial use and electrical generators.
Managing heat transfer in these small scale solutions currently employ more modern, “compact” industrial heat exchangers like welded plate or printed circuit heat exchangers (PCHE). These are robust units also made using traditional manufacturing or diffusion bonding, where layers of etched metal are compressed and heated until they fuse into a single block with the material’s original strength and characteristics.
These heat exchanger technologies have been in use for a long time and have been refined over the years. PCHE heat exchangers, for example, whilst being comparatively ‘new’ have been in production for approximately 40 years. Certification standards, processes and data on durability are therefore well defined, providing strong data on performance, design life, reliability and servicing requirements.
Manufacturing time and cost for many of these solutions may be high, leveraging bespoke machinery with high tooling costs. They may be comparatively bulky and heavy for mobile or space-constrained applications.
Additive Manufacturing in Energy heat exchangers
Driven by the need to be more efficient and developing more sustainable practices, energy companies are fast exploring the benefits and solutions offered by AM technology and understanding both the challenges and opportunities this technology can offer in its current and near-future state.
Benefits of additive manufacturing for energy heat exchangers
The core benefit that AM offers is the capability to intensify the heat transfer process and provide new approaches to maintenance processes. New freedoms of design, bespoke packaging and the opportunity to leverage novel materials and processes are delivering higher performance and lower pressure drop, also enabling reduced volume, size and weight. Monolithic parts and the promise of distributed manufacturing drive potential reductions in operational expenditure associated with large assets and lower supply chain and supplier risks.
- Performance – the ability to design a wildly different and “exotic” core geometry that is free from the constraints of traditional tooling means the core geometry can create a more effective transfer of heat for a given set of fluids. Small scale fins and turbulators can be printed as inherent elements of the channels reduce unwanted fluid boundary layers whilst optimizing pressure drop (more explanation here). With accurate simulation of the fluid properties, the geometry of a given core can be varied to suit the specific boundary conditions of the application, and even vary within the core to optimize for the changing properties of the fluids being cooled. Multiple fluids can be incorporated into a single core with comparative ease, further increasing efficiency within a system.
- Bespoke dimensions and packaging – AM also provides improved freedoms regarding the overall dimensions and shape of a heat exchanger. A heat exchanger can be contoured to accommodate other system components, space constraints or can be developed as cartridges or inserts to fit into traditionally manufactured housings. Radical shapes also come with very minimal impact on the end of cost of the item, although performance may be impacted.
- Light weighting, reduced volumes, reduced size – highly efficient heat exchange implicitly results in less bulk, weight and material use. Entire heat transfer loops may be removed from processes where significant efficiencies in constrained volumes or multi-fluid heat exchangers can be employed.
- Monolithic parts– a single part may be produced with a single input material reducing the BOM. This has the potential to reduce or remove inherent failure points at joins, although the manufacturing process also requires careful control of calibration, parameters and design to avoid other challenges.
- Novel materials and processes– Research into new materials with complex micro and nano structures is yielding many applications across the energy sector (see article by Li Zhang et al for an overview). More immediately for heat exchangers, new alloys with high thermal conductivity, low weight and high durability are being investigated.
- Distributed manufacture – An additively manufactured part has the potential to be “printed” close to or at the site of use, without relying on highly specialized tooling . Whilst significant complexities are currently involved – such as post-processing requirements, machine calibration and IP protection – this has long term potential to reduce transport costs, inventory levels, supply chain risks and further enhance serviceability in the long term.
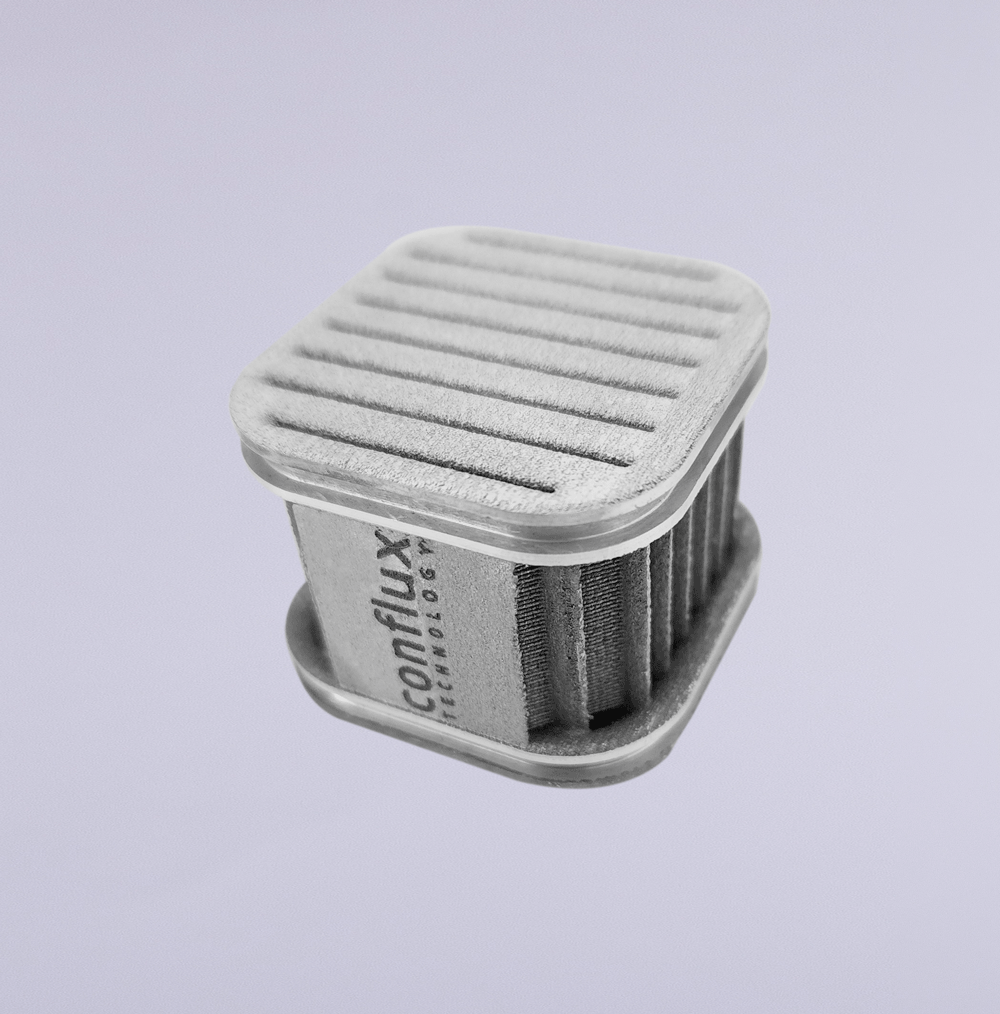
How do you create large scale heat exchangers using additive manufacturing?
Additive manufacturing is still developing the appropriate techniques to “print metal” at the large scales required. There are two paths – enlarging machines and creating modular arrays.
But how big can you print a metal heat exchanger? To be able to print the small scale features, laser powder bed fusion (LPBF) machines are generally used. The footprint able to be printed on commercially available machines is currently limited to sub-1m3. For example, AMCM https://amcm.com/ customizes EOS machines, and has developed a machine that is able to 450 x 450 x 1000 mm. Other technologies such as Directed Energy Deposition promise larger volumes with lower resolution (example).
To satisfy the needs of this situation we look to the design and consider a set of modular components.
Modular Arrays
A modular array is a set of parts of cells designed to join to form a larger unit. A modular design offers a host of potential benefits; flexibility, configuration, scaling, customization of individual parts, evolution of design, single unit replacement and serviceability.
Modularity in heat exchangers is not a new concept –plate, PCHE and welded heat exchangers all support modularity via layering of repeating elements within a unit or by series or parallel linking of units.
As well as allowing for scaling beyond a single unit, modules can be reconfigured, or individual units can be removed and maintained without having to shut down the entire process (say by closing a set of valves). When considered large scale energy production the appeal of being able to maintain constant production is of high value given the financial gain.
Disadvantages include the potential for leak points at joins, 'dead areas' at joints that lower the efficiency for a certain volume and higher complexity and costs. Reliable joining of modules without compromising integrity or over engineering is a challenge shared by all heat exchangers, and for applications suited to AM where compactness is critical, this is a bigger issue.
Additively manufactured heat exchanger modules are following similar paths, therefore have design and manufacturing precedents to follow: incorporating one or more AM cores in traditionally manufactured casings following similar linking strategies, or leverage direct joining of modular elements that are a single AM part. Comprehensive data on best practice and the effects of welding AM materials is not yet widespread.
Certification and standards are evolving – a critical step in AM adoption
The AM process flow including the system/platform, process and material need to be qualified to a certain level to produce certified parts that meet standards, design specifications and end use performance targets.
It is clear that qualification and certification are two topics that are critical for increasing the adoption of AM heat exchangers, and International standards are emerging for the AM industry. A growing number of specific parts, processes and facilities are being certified for high value applications, especially in aerospace.
There are many other product specific standards under development by various bodies such as ISO, ASME and ASTM that could be applied more broadly across other industries. For example, the National Aerospace and Defense Contractors Accreditation Program (NADCAP) now includes a certifiable standard (AC7110/14) specifically for laser and electron beam powder bed fusion.
As new applications are developed in the energy sector through research and commercial endeavor, additively manufactured heat exchangers will undoubtably find a growing niche in the energy market where compact, high value heat transfer efficiencies are required.